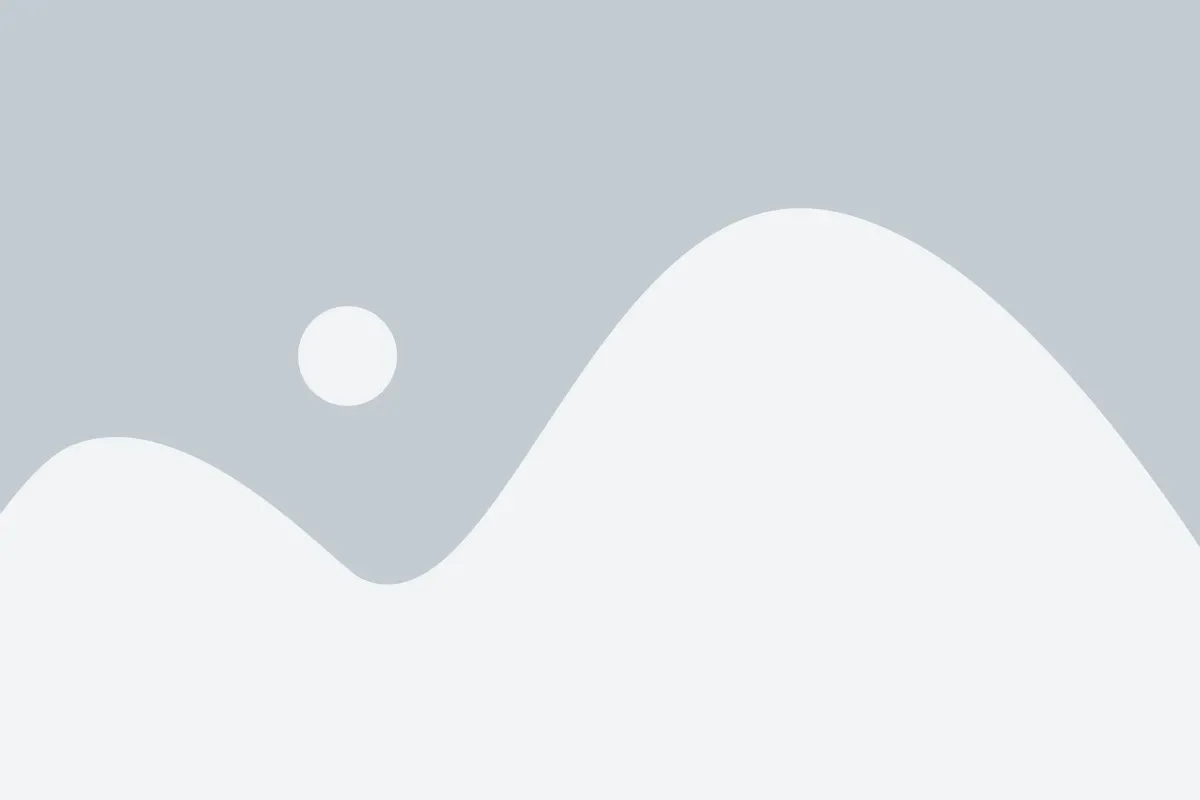
Biological effects of “light”
Dr. rer. nat. Ralf Neurohr, Spiesen-Elversberg
DETERMINING THE POSITION (LIFE SCIENCES IN THE 20TH CENTURY)
The tremendous advances in the (natural) sciences at the end of the 19th and 20th centuries, against which any reasonably serious or significant scientific discussion should be regarded, have profound consequences and the implications of such advances are not always given due consideration even by those involved in the life sciences. This situation, although unsatisfactory, is quite understandable: in this context, tremendous advances not only mean an ever deeper penetration of our knowledge into molecular and subatomic fields, i. e. into chemicophysical relationships and principles, but also an increasing spread in the range of “fringe areas” which are brought in as experimental aids in research and teaching or to clarify and interpret experimental results.
This situation has inevitably led to diversification and specialisation with the development of individual specialised sub-disciplines, generally accompanied by a not insignificant process of selfrestriction.
Thus, for example, in the course of the inevitable trend towards specialisation, the disciplines of biochemistry and microbiology first developed where chemistry and biology intersect. From them ultimately emerged the interdisciplinary field of molecular biology which, in turn, gave us highly specialised, predominantly applied disciplines such as genetic engineering and biotechnology which are both currently the focus of such intense public interest.
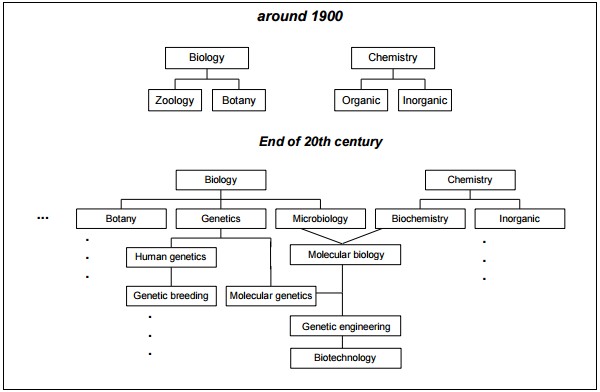
However, we are not so much concerned here with the ongoing public debate embracing ethical and philosophical issues and which cannot, at least in part, be conducted using arguments based on the natural sciences; we are more concerned with the paradigmatic aspects of contemporary life sciences characterised as these are by a molecular approach.
REDUCTIONISM VS. VITALISM
From a historical perspective, modern biology is therefore derived from an increasingly sophisticated material characterisation of living systems in which molecular, i. e. chemical, laws and mechanisms are used to describe the interactions between the components of the system. A pointed representation of this view can be found, for example, in the preface to the biology textbook edited by Czihak, Langer and Ziegler (Springer Verlag 1990):
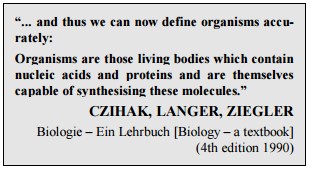
This interpretation of organisms as molecular machines (statements generally relate to cellular units) refers to the extraordinarily successful reductionist approach in the natural sciences whose declared aim is to provide an integrated description of nature and its phenomena. This principle can be outlined in simplified form as follows: all matter consists of a number of fundamental constituents (so called elementary particles) from which even such complex structures as molecules, biopolymers or cells are made. All interactions between these constituents can be attributed to just 4 fundamental forces which are responsible for the way the elementary particles behave in relation to each other, the development of complex structures and their interaction at the macroscopic level.
Although this approach appears quite reasonable and plausible even to non-scientists, there have been recurring opposing views in the form of so called vitalistic theories which proclaimed the existence of their own laws and/or forces for living systems which could not be reduced to known interactions and chemicophysical facts. This thesis in turn appears somewhat irrational and accordingly has repeatedly aroused fierce criticism, sometimes leading to severe clashes between supporters of vitalistic ideas and advocates of a reductionist description of nature. In the collection of essays on the subject by renowned scientists from the fields of physics, chemistry and biology which he has edited, Bernd Olaf Küppers, a student of Nobel Laureate Manfred Eigen, has reduced this conflict to the succinct formula
life = physics + chemistry?
Through publications such as “Der Ursprung biologischer Information” [The origin of biological information] and “Molecular Theory of Evolution”, he himself has not only provided a clear declaration on the reductionist approach but also an example of the frequently encountered dogmatic overinterpretation of the reductionist thesis.
Strictly speaking, reductionism is, and was, often “misunderstood” as molecular reductionism, which can partly be explained by the enormous achievements and successes of basic research in molecular biology and supported impressively by the quote from Czihak, Langer and Ziegler. However, this viewpoint restricts, for no valid reason, the original idea of an integrated description of nature to a certain level of description – namely to the level of molecular structures and their interactions. In its more general form, however, a reductionist approach should not a priori exclude from consideration other levels of description, such as that of primary physical effects. Extending the horizon in this way – beyond the molecular level – might actually yield explanations which cannot, or are very difficult, to obtain with purely molecular models, which generally relate to closed systems under equilibrium conditions.
NON-EQUILIBRIUM THERMODYNAMICS, OPEN SYSTEMS AND INFORMATION
Biological systems can be described as thermodynamically open systems per se which can only maintain their internal order and outwardly directed activities through a constant supply of matter and energy. Substances high in energy with a high degree of order are supplied through food and transformed via metabolic processes. As a result, energy and order in food are used to build up and maintain endogenous structures and substances with a low degree of order are excreted as the end products of metabolism. Without this permanent flow of energy and matter the system would soon collapse and disintegrate into its components, corresponding to a relapse into thermodynamic equilibrium.
Ilya Prigogine coined the term dissipative structures for these open systems, far removed from a state of equilibrium, which develop characteristics in the simple model system which display astonishing parallels with the self-organising characteristics of living systems. In conditions without equilibrium (e. g. maintenance of a concentration gradient), even simple reaction mixtures of just a few inorganic components develop spatial or temporal response patterns which clearly prove collective behaviour and self-organisation on the part of the participating molecules (which, in equilibrium, normally act independently).
Interestingly, the spatial-temporal patterns observed are determined by the initial or boundary conditions under which the reaction system starts. Thus it has a kind of memory function, unlike equilibrium systems where any information about the initial situation is lost the nearer they get to the final state. Prigogine himself summarised this fact as follows:
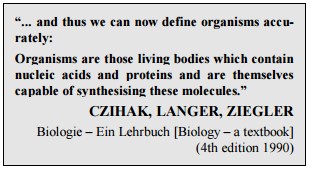
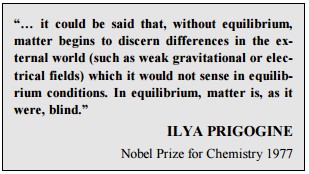
This forms a basis for describing biological systems which, without contradicting the reductionist thesis, extends beyond the molecular reductionism referred to earlier. The characteristics of biological systems listed by many vitalists as arguments to support vitalistic theses, such as selforganisation, communication or cognitive abilities, certainly do not require controversial or speculative molecular models but can already be deduced from the discovery of non-equilibrium thermodynamics.
An impressive indication of the actual significance of this fact was provided by the research group led by the physicist Fritz Popp who made the following observation concerning the emission by living systems of weak light signals known as ultra-weak photon emissions (UPE): if the spectral composition of the emitted light is tracked for a sufficient length of time, the resulting calculated spectral distribution of occupation probabilities in the biological system can be seen to differ markedly from that expected in a state of thermodynamic equilibrium. With smaller wavelengths this difference reaches several orders of magnitude corresponding to colour temperatures of several thousand kelvin. Biological systems therefore appear to have an (admittedly weak) photon field, capable in principle (when far removed from the state of equilibrium) of providing any energy and thus intervening in the molecular process of the cell. According to Popp’s ideas, this field has further cooperative properties such as coupling excited states and cooperative memory functions which predestine it precisely as a central mechanism for regulating and communicating.
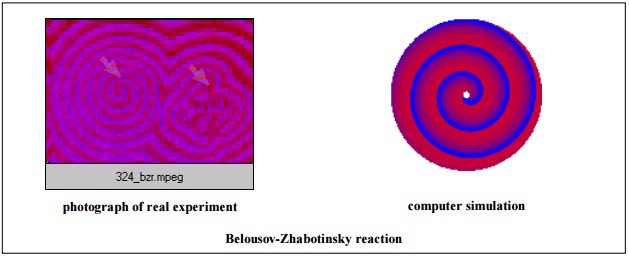
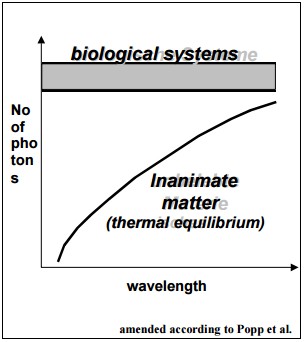
Even if Popp’s theses are not exactly widely accepted, the phenomenon of ultra weak photon emissions still provides a useful basis for investigating biological systems beyond the molecular level of description, which is extremely difficult if, for example, the coherence of the body’s entire regulatory system is to be described. The following two facts can serve as an example of this:
If we consider a single protein molecule, its socalled tertiary structure (spatial folding of the amino acid chain), which is vitally important for correct biological function, has hundreds, if not thousands, of competing states, some of which at least are of equal energetic rank at body temperature. This means however (provided the system is in a state of equilibrium) that only a fraction of the protein is ever in its active and thus useful form, while the species’ remaining molecules would “swim around in the cell like useless ballast”. This is not the case however and we observe instead that, following their synthesis, most proteins occupy their active structure in an extremely targeted fashion and maintain this even despite thermal impact. No molecular stabilising mechanism has so far been described.
The phenomenon of the biological clock creates even more problems for the formulation of a universally valid molecular model. Probably the best known form of this phenomenon, circadian rhythms at each individual’s organisational level, moulds our entire daily routine. However, alongside this, rhythms spanning longer periods (circadian rhythms, circannual rhythms) have also been discovered, as well as ones spanning shorter periods (circatidal rhythms). Based on the correlation with external cycles such as daily and seasonal changes, cycles of the moon or changing tides it was generally first assumed that the periodic processes in biological systems are controlled by external signals, i. e. are passive in nature. Detailed investigations then revealed however that this is only half the truth, as when sealed off from all known external trigger signals, all the systems studied still display a so called free running rhythm. The endogenous character of these freerunning rhythms is particularly clear through the fact that their periods are not exactly the same length as those of external rhythms but may be shorter or longer, to varying degrees. External rhythms, such as the change from day to night, only perform a trigger function in so far as they serve to synchronise the freerunning internal clock. Various molecular models have already been put forward for discussion as mechanisms for this internal clock and for its coupling to external timers without, however, any having been finally accepted. Instead, experimental results suggest the conclusion that the internal clock is a physical process which can be described (at least in theory) by a system of coupled oscillations which responds to many different chemical and physical trigger signals.
PHOTON EMISSIONS BY BIOLOGICAL SYSTEMS
If it were possible to demonstrate a connection between phenomena similar to this example, which are not yet fully understood, and the photon field characterised by UPE, it would be obvious that these light signals or long trajectory electromagnetic couplings in general should be accepted as another possible mechanism within the range of biological regulatory mechanisms. Therefore, we should examine the phenomenon itself in more detail as well as some extremely interesting experimental findings.
PHENOMENOLOGY OF UPE
This is a spontaneous light emission of extremely low intensity, only slightly above the noise level of the cooled, highly sensitive measuring apparatus at around 20 counts/sec, which (depending on the detector’s quantum yield) corresponds to around 50 to 100 photons per second. Because of this low intensity, many experimental investigations use a flash to help excite the system externally, whereby the decaying of the photon field described as delayed luminescence is investigated. Due to the considerably improved signal to noise ratio, this produces clearer results which can therefore be interpreted better.
The only reason for attaching any significance to this as more than a weak light emission is some of its characteristics which indicate that this is a coherent radiation which cannot be attributed to one or more independently emitting sources.
The emitted photon stream appears to derive from a highly ordered coherent field, as seen in laser light, for example, – quite unlike the stochastic emission from thermal light sources such as light bulbs or the spontaneous emissions of excited co-reactants which might also be expected from cells due to metabolic defects (in that case with a higher intensity though). The decaying of delayed luminescence can serve as an example here: if independent emitters were involved here, a very rapid exponential decay within less than one second would be expected based on the short life of molecular excited states. Instead we observe a hyperbolic decay curve which, even after over one minute, is still well above the spontaneous emission level. Both are clear indications of the coherence of the radiation and the coupling between the responsible excited states.
This example highlights another frequently observed quality of delayed luminescence, a correlation between decay behaviour and the “physiological state” of the test system. Two similar samples were used, one of which was contaminated with germs (curve 2, red, on next page), while the other was unimpaired (curve 1, blue). Closer inspection of the two signals shows that the contaminated sample obviously decays quicker at a higher initial intensity than the unimpaired sample. This behaviour, which was observed in numerous other cases, can be explained with the model of a universal light store whose effectiveness is closely linked to the general state of the system. The better the general state, the more effectively our hypothetical photon store operates, reflected in the form of low intensities and long decay times. This interpretation also accords with the fact that the spontaneous emission is so extraordinarily low – an intact system should have a large light storage capacity and display as low a “leakage flow” as possible.
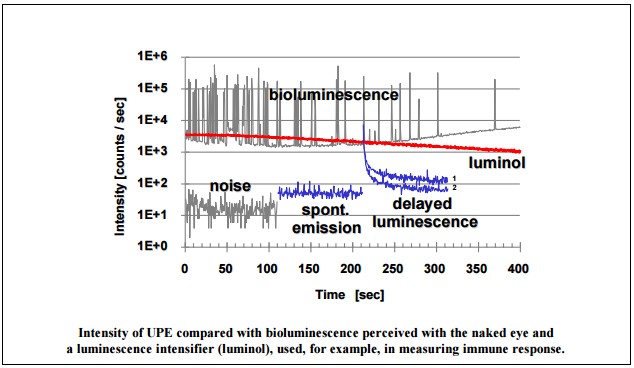
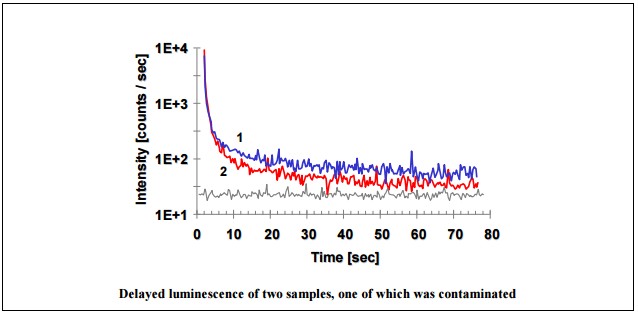
Although this is an abstract model for descriptive purposes, it is this correlation between ultraweak or delayed luminescence and the relevant physiological states of the systems studied, which makes it appear worthwhile considering this phenomenon further.
DELAYED LUMINESCENCE IN SEEDS
If, for example, delayed luminescence in seeds is tracked over the period of one year, a signal is obtained which rises from autumn in the form of a saw tooth oscillation which is apparently superimposed by higher frequency oscillations. The signal reaches a maximum in spring in the form of a clearly defined peak to then fall again markedly, although not reaching the level at the start of the previous year.
Statistically, the path of this signal can be broken down into a rising linear component and a series of fairly regular oscillation components which will be examined in more detail below. The clearly defined spring maximum first suggests the conclusion that we are dealing here with an annual fluctuation. We cannot prove this however as only one observation period is available – an observation period of at least 2 years would be necessary to do this.
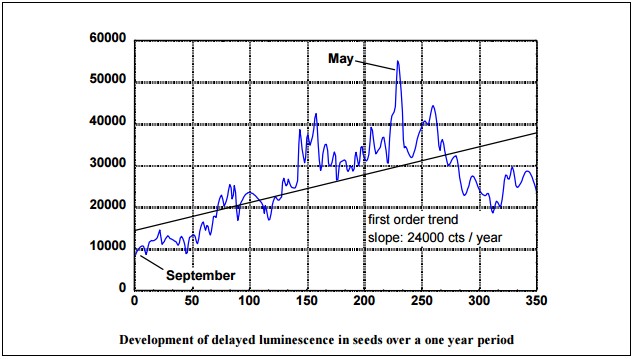
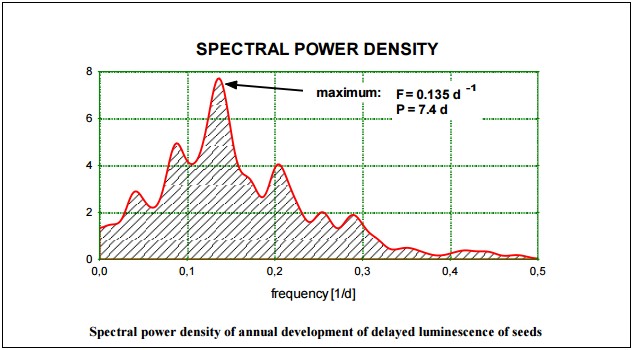
All high frequency parts of the oscillation can be recorded however using a Fourier transform. The resulting spectral power density shows that the higher frequency parts definitely oscillate with a preferred period of just over 7 days. Any known external influences on the luminescence of seeds, e. g. fluctuations in atmospheric humidity, etc. can be ruled out as an explanation for this periodic behaviour.
We are thus faced with the problem of interpreting this unpredicted experimental result in a meaningful way. This is possible however if we use publications on biological rhythms of seeds as a comparison.
SWELLING AND BIOLOGICAL RHYTHMS
The Belgian research group working with Spruyt et al. has devoted considerable attention in several publications to the rhythmic fluctuation of the water uptake of swelling seeds and discovered the following:
There is a typical annual course for water uptake in the initial hours of swelling which can be verified over several years in virtually all types of seeds studied.
A higher frequency oscillation with an amplitude of around 20% of the annual amplitude is superimposed over the annual path of this fluctuation. The period of this shorter oscillation obviously follows the 4 phases of the moon and is therefore 7.4 days long.
Spruyt et al. consider these findings closely linked to the phenomenon of the biological clock, yet do not speculate as to possible underlying mechanisms. The swelling rhythms observed are essentially used as “pointer” to the biological clock to look into other problems in this connection.
Based on the agreement between rhythmic swelling and delayed luminescence, we are now at least able to interpret these as a pointer to the biological clock, whereby this pointer has a clear advantage over other experimental methods when measuring the biorhythms of seeds: it is a non-invasive method, which does not damage the seeds in any way and, in particular, does not adversely affect their germinative capacity.
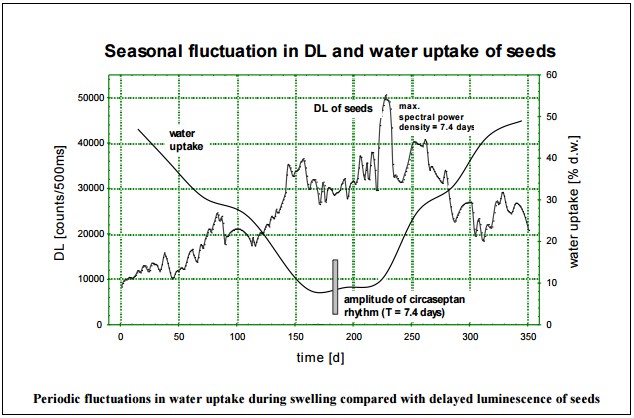
The results also enable a new working hypothesis to be formulated which takes us beyond the initially criticised molecular character of previous regulatory models: in view of the unanswered questions surrounding the mechanism of the internal clock, it is also conceivable that a coherent field (such as the photon store mentioned earlier) may itself function as a biological clock. A field like this interacts with many of the cell’s components and can thus react to a large number of timer signals, as is required based on existing knowledge. In addition, it is recorded that, in a dry biological system, such as seed, most of the molecular mechanisms previously suggested are unable to function due to the low level of hydration. This applies particularly to those models which operate with diffusion gradients or the periodic exchange of signal transmitters between the cell nucleus and cell membrane.
This does not in any way question or discredit the ideas of molecular regulation, yet at least it makes it seem convincing that, beyond molecular reductionism, there are still discoveries to be made which could very much enrich our conception of the function of biological systems.